Carbohydrate Background
Carbohydrates are a class of molecules as diverse in structure as they are in function. While we may be familiar with the carbohydrate section of a nutrition label that quantifies starches, fibers, and sugars, we are becoming increasingly aware of more subtle differences between them — such as the distinction between glucose, fructose, and sucrose, which are highlighted in the debate about corn sugar, high-fructose corn syrup, and table sugar, respectively.
Beyond our dietary intake, carbohydrates play important roles in energy storage and metabolism as well as cell structure, cell-to-cell communication, and cellular-level defenses.
Carbohydrate Chemistry
What is a carbohydrate?
Carbohydrates are also known as saccharides (Greek for sugar). From a basic structural standpoint, a carbohydrate is considered to be a “hydrate of carbon,” which basically means that for each carbon molecule, there is also one molecule of water. For instance, when considering the molecular formula for glucose, C6H12O6, we can rearrange it to C6(H2O)6 to indicate 6 carbon atoms and 6 water molecules. Carbohydrates can exist as simple sugars (monosaccharides), or as more complex sugars like sucrose (disaccharide) or starch (polysaccharide), which involve the polymerization of monosaccharide units.
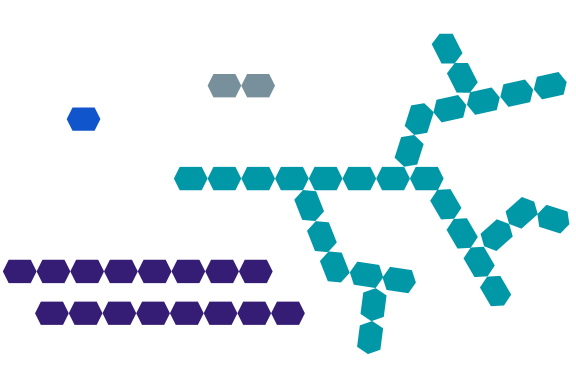
Typical sugars can be monosaccharides (blue) or disaccharides (gray), while carbohydrates are polysaccharides that can be branched (teal) or linear (purple)
Simple Sugars: Monosaccharides
Monosaccharides are the single sugar molecule building blocks. These often consist of 5-7 carbon molecules bonded together, though they can be assembled from as few as 2 carbons, or even 8 or more (these larger sugars are often unstable). Hundreds of different monosaccharides are known, and functions based on different locations and geometries of one carbonyl carbon (C=O) and several hydroxide (-OH) groups. If the monosaccharide has the carbonyl on the first carbon, then it will contain an aldehyde group (left structure), whereas a carbonyl on the second carbon makes a ketone group (right structure).
Monosaccharides often exist as ring structures due to a reaction between the aldehyde or ketone on one end of the carbon chain with a hydroxide at or near the other end, forming an ester-type linkage that is called a hemiacetal or hemiketal, respectively. This is a reversible reaction, and occurs rapidly and spontaneously (no enzyme needed) for most sugars when in aqueous environments. However, the cyclized (ring) form is usually the more prevalent configuration for sugars that form 5- and 6-membered carbon rings.
Four depictions of beta-D-glucopyranose (glucose) are shown above. The leftmost drawing is called a Fisher projection and draws a linear sugar molecule with the hydroxide geometries depicted by whether they project to the left or right of the vertical carbon backbone. The second drawing shows the process of cyclization between the carbonyl carbon (pink aldehyde in the first picture) and the penultimate (second to last) hydroxide. The formed hemiacetal is highlighted in blue.
The third drawing shows the cyclized sugar as a Haworth projection, where hydroxides pointing above the ring extend vertically upward from their carbon while hydroxides pointing below the ring extend vertically downward from their carbon. The final, fourth structure is the same sugar shown in a chair conformation, most similar to the three dimensional shape of the sugar. In both the Haworth projection and the chair conformation, the thicker line in the ring depicts the side of the ring coming out of the page toward you and the blue shape highlights the hemiacetal.
Structure Dictates Function
Different monosaccharides — glucose, fructose, etc. — may vary in the number of carbons, as well as the orientations of the -OH groups coming off of each carbon atom. Each particular set of orientations in 3-dimensional space creates a unique molecule, meaning sugar molecules can come in many different flavors — literally. The three dimensional shape of the sugar molecule affects how it interacts with taste receptors and metabolic enzymes so different sugars vary in sweetness and digestibility. For example, humans perceive fructose to be much sweeter than glucose, which is why it is added to corn sugar to create “high fructose corn syrup.”
Another example of how small molecular changes impacts function lies in DNA and RNA. DNA contains deoxyribose sugar, and RNA ribose sugar in their backbones — both deoxyribose and ribose contain 5 carbons, but differ in the absence (deoxyribose) or presence (ribose) of single oxygen atom. This seemingly small difference between the two sugars changes the stability and chemical reactivity of the different forms of genetic material.
Complex Sugars: Disaccharides to Polysaccharides
Monosaccharide building blocks can also bond together to formed from a pair of sugars (disaccharide) or a long or even branching chain of sugar units (polysaccharide). What we think of as table sugar (sucrose) is actually a disaccharide formed from the linkage of one glucose and one fructose, with an ester bond between them (highlighted in green). The geometry of the linkage formed between sugars is also important for metabolism.
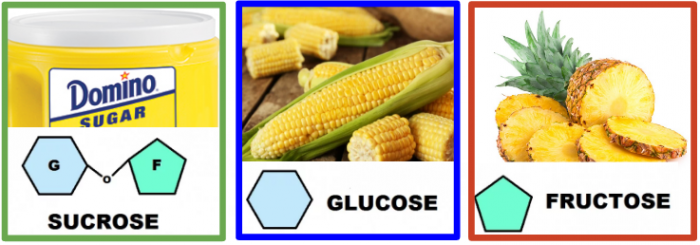
Sucrose (a disaccharide) is comprised of one glucose (monosaccharide) and one fructose (monosaccharide) covalently bonded together, changing our perceptions of sweetness along with our metabolism of the sugars
Another familiar disaccharide is lactose, which is found in dairy products. The specific linkage between the two sugar rings in lactose is digested by a unique enzyme called lactase, which is nots expressed in many adults populations throughout the world, causing wide-scale lactose intolerance.
The linkage between two sugar molecules is an example of dehydration synthesis, the same type of reaction as used to combine amino acids to make a protein. Also similarly, the reverse reaction, hydrolysis, breaks sugars back into monosaccharides.
Just as dehydration synthesis occurs repeatedly between subsequent amino acids to make proteins, this reaction occurs repeatedly to make long chains of sugars. This process is known as polymerization, where the units of assembly (in this case monosaccharides) are called monomers and the product of the polymerization reaction is a polymer (an oligosaccharide or polysaccharide). Unlike making proteins, there are many hydroxide (-OH) groups on a sugar that can participate in dehydration synthesis, so the polymers can be linear chains or or branched structures.
Polysaccharides are longer sugars and are most commonly used as energy storage in different organisms. Plants store energy as starch and cellulose, which are polymers of glucose. Humans can break the sugar-to-sugar linkages within starch, and thus we call this a digestible sugar. In contrast, we cannot break the linkages in cellulose and so we call this dietary fiber. Humans store energy as polymers of glucose called glycogen, which are heavily branched sugar structures.
Even within the categories of starches and fiber, there are many specific carbohydrate structures. In much of our food, starch is comprised of two main molecules: amylose and amylopectin. Amylose has a linear structure while amylopectin has a branched structure. These structural differences are important for the digestion of different starches, where enzymes hydrolyze sugars from the ends of polymer chains. Thus, the more highly branched amylopectin structure can be more efficiently digested than linear amylose.
Why Are Carbohydrates Important for Life?
Glucose Is An Ancient Source Of Energy
While there are multiple theories on the origin of biological life on earth, the exact mechanism for how life originated is still a puzzle that has yet to be solved. However, evidence does suggest that it took place approximately 3.8 billion years ago, at a time when the earth’s atmosphere contained little or no free oxygen (Precambrian). Consisting primarily of CO2 and N2, this primitive atmosphere provided the reducing conditions that allowed for the spontaneous formation of organic molecules, thus creating the “primordial soup” that set the stage for the first single-celled organisms.
Because the first single-celled organisms arose in a sea of organic molecules, they were able to obtain energy directly from their environment. However, this had limitations — given the rapidly changing ecological landscape of early earth, it became important for these first lifeforms to develop a more sustainable mechanism for generating the energy needed to grow and replicate. This process, which is central to all organisms to have ever existed on our planet, focused on the production of the most conserved molecule of all time: adenosine 5’-triphosphate, otherwise known as ATP.
As ATP evolved to become the driving force for nearly all energy-requiring cellular functions, so did the enzymatic cascades responsible for producing it. We believe that glycolysis — the cellular process of using glucose to produce ATP — was the first ATP-generating mechanism to arise. Evidence to support this includes the fact that it is present in all organisms and that it occurs in the cytosol of cells (does not need organelles). Furthermore, the enzymes involved in glycolysis display a significant level of evolutionary conservation across all known organisms, at the levels of function, structure, and sequence homology.
The Biochemistry of Glucose Metabolism
Glycolysis, which literally translates to “sugar splitting,” consists of a ten-enzyme cascade, where glucose (6 carbons) is broken down into 2 molecules of pyruvate (3 carbons). The process of glycolysis can be divided into 2 phases: the energy investment phase, which requires 2 molecules of ATP, and the energy output phase, which yields 4 molecules of ATP. This process, which nets 2 molecules of ATP, also results in the reduction of 2 NAD+ to 2 NADH. In addition to providing the cell with energy in the form of ATP, the reduction of NAD+ to NADH will provide an additional benefit for cells through its ability to donate electrons (reducing agent).
Once the process of glycolysis was established and early microbial cells had a mechanism to utilize the organic molecules around them to generate ATP (energy), the next step in the evolution of metabolism is thought to be the emergence of photosynthesis. By providing these early organisms with the capacity to convert sunlight into energy, photosynthesis released these cells from being reliant on the “primordial soup” to function, giving them energy independence. The by product of photosynthesis, O2, had the added benefit of changing the earth’s atmosphere, potentially giving rise to oxidative metabolism — a process where oxygen is used to convert carbohydrates into energy (as opposed to glycolysis, which can occur under anaerobic conditions).
Fast forward about 2 billion years, and glycolysis became fully and efficiently integrated with oxidative pathways. When oxygen is present, the 2 molecules of pyruvate generated as a result of glycolysis can be shuttled into the Krebs Pathway (also known as the Citric Acid Cycle), followed by the electron transport chain — both of which occur in mitochondria in eukaryotes. In total, the oxidation of glucose into carbon dioxide (cellular respiration) yields an approximate total of 32 molecules of ATP. With an efficiency that is more than an order of magnitude better than glycolysis alone, the process of cellular respiration allowed for an explosion of life forms, giving rise to the biodiversity seen throughout evolutionary history.
Metabolism of Carbohydrates in Humans
Carbohydrates and Human Nutrition
Just like the ancient microbes that first used sugar to produce ATP, our bodies also rely on sugar to generate energy. On the cellular level, glucose undergoes transformation into CO2 and ATP via oxidative metabolism (glycolysis → Krebs Cycle → electron transport chain). However, when looking at carbohydrate metabolism at the whole-body level, things become a bit more complex.
Yielding about 4 kcal/g, carbohydrates are the primary fuel for many cell types in our body. For instance, because of its large size, the human brain has unusually high metabolic costs, and is one of the major consumers of glucose — it requires about 120 grams of glucose per day, or about 60% of the body’s resting metabolic rate. So how do the carbohydrates in our food, like those in our egg sandwich, make it to the cells that use them?
The digestion of carbohydrates begins in our mouths. When we eat food containing starch (long chains of glucose), salivary amylase, which is present in saliva, starts to break down the starch into smaller disaccharides units, such as mannose. Once the carbohydrate is swallowed, it enters the stomach, which has a pH of about 1-2. This acidic environment deactivates the salivary amylase and carbohydrate catalysis is paused.
Upon leaving the stomach and entering the small intestine — a more suitable environment for digesting carbohydrates (pH 7) — the pancreas is stimulated to secrete pancreatic amylase, which continues to act on the starch remnants. In addition to pancreatic amylase, the lining of the small intestine also secretes a variety of enzymes that break disaccharide units down into monosaccharide units:
- Maltase: converts maltose into 2 units of glucose
- Sucrase: converts sucrose into fructose and glucose
- Lactase: coverts lactose into glucose and galactose
After starch has been broken down into simple sugars, the individual monosaccharide units cross from the digestive tract into the bloodstream. More specifically, glucose, galactose, and fructose absorbed by intestinal cells (where some fructose is converted into glucose). From there, the sugars are transported into the bloodstream, and travel via the portal vein to the liver. Depending on the needs of the body, the liver will act on the sugars in one of three ways:
- Convert all monosaccharides to glucose and release into the bloodstream — this provides other organs with fuel
- Polymerize glucose into glycogen — the storage form of glucose found in the liver
- Convert excess sugar into fat
Which path glucose takes must be tightly controlled. Any variations in this could lead to major organ damage or even death. Luckily, humans (and many other animals) have developed an intricate system to coordinate the utilization and storage of glucose in our bodies.
Metabolic Regulation of Glucose in Humans
The amount of glucose that is present in our bloodstream at any given time must be tightly regulated. In general, the average plasma (bloodstream) glucose level should fall between 70 – 120 mg/dl for a 24 hour period. Major deviations from this can have serious consequences — so how does our body ensure that glucose levels are controlled?
The answer is an intricate orchestration of sensory cues and hormones involving multiple organ systems. But before we get into the symphony that this orchestra plays, it is important to first define who the actual players are:
Insulin: Secreted by the pancreas, insulin is a peptide hormone that promotes glucose removal from the blood by stimulating organs (like skeletal muscle or the liver) to absorb glucose and store it.
Glucagon: Also secreted by the pancreas, this peptide hormone has the opposite effect of insulin — it promotes the release of glucose from organs into the bloodstream.
Glycogen: This is a polysaccharide made up of glucose units. It is highly branched, and is made and stored in the liver and muscle. It has a similar structure to amylopectin, which is found in plant starch.
Liver: The liver is the major metabolic regulatory organ in our bodies. It is the storage site for the majority of glycogen, and provides approximately 90% of the glucose that is not derived directly from our diet.
Skeletal Muscle: This organ system is critical when it comes to providing a rapid response to high glucose — it can quickly absorb glucose from the blood, and help manage any spikes in glucose levels. Here, glucose is stored as glycogen, and it predominantly used to satisfy the energy requirements of the muscle itself (instead of being released into the bloodstream).
Pancreas: This endocrine organ secretes insulin and glucagon.
When we eat carbohydrate-containing food, it causes a rise in blood sugar (plasma glucose). The pancreas will respond by secreting insulin, which promotes our organs, primarily the liver and skeletal muscle, to absorb glucose and store it as glycogen. However, when we have low blood sugar (hypoglycemia), the pancreas will secrete glucagon, which will tell the liver to break down glycogen stores, and release glucose into the bloodstream for utilization.
If this process is disrupted in any way, it can have serious consequences for health. Too much glucose in the blood could be very toxic to cells and organs, which is why the process of glucose metabolism is tightly regulated. However, due to a culture of poor dietary choices along with genetic influences, a disruption in this system is extremely common — this statement can be quantified by looking at clinical records. The rate at which people are developing type 2 diabetes is astounding, with nearly 2 million new cases per year in the United States alone (American Diabetes Association). What are the molecular mechanisms underlying this epidemic?
A poor diet, sedentary lifestyle, and an individual’s genetics all influence the efficacy of glucose metabolism in humans. When there is a prolonged increase in blood sugar levels, as well as prolonged increase in circulating free fatty acids, the metabolic system controlling glucose homeostasis becomes taxed, reducing the natural ability to coordinate a response. Over time, muscle cells will stop responding to insulin, becoming insulin resistant. When insulin resistance in muscle occurs, blood sugar levels can skyrocket, and affect other organ systems, including the pancreas, and thus, the ability to produce insulin.
While the inability to control blood glucose levels is a defining phenotype of both type 1 and type 2 diabetes, they are very different in their pathologies. In type 1 diabetes, which is actually an autoimmune disease, the body wages an immune response against pancreatic beta cells, essentially destroying them. Because beta cells produce insulin, the immunological attack on these cells leads to the inability to produce insulin. This is why people who have type 1 diabetes must inject synthetic insulin on a regular basis (or wear an insulin pump). On the other hand, type 2 diabetes is a disease that is developed over time. In this case, the pancreatic beta cells are (at first) able to produce insulin. However, the ability of muscles to respond to insulin and actively transport glucose out of the bloodstream, becomes hindered.
More specifically, the Glut4 transport protein, which normally lives on the surface of muscle cells, becomes ineffective. Over time, the high circulating blood glucose levels (in addition to free fatty acids and other inflammatory factors) will continue to affect organ systems, and ultimately lead to death of pancreatic beta cells, rendering an individual unable to produce insulin altogether, compounding the effects of reduced or no glucose transport into cells.
Interestingly, there are some studies that suggest specific foods will have different physiological responses in terms of glucose homeostasis in our bodies. These responses can be predicted by understanding the glycemic index and the glycemic load of foods. The glycemic index is a measure of how a specific food will affect a person’s blood glucose levels. This measurement takes into account the total rise in blood glucose, but it may not inform the actual rate of this rise. Nonetheless, it is a useful tool in understanding how our body breaks down carbohydrates. There are multiple factors that influence the glycemic index, including starch structure, fiber content, manufacturers processing, and other macronutrients present (like fats).
When the glycemic index of a specific food is known, we can use that to determine the glycemic load of a food. The glycemic load is a quantitative prediction of how much glucose will appear in the bloodstream as a result of eating a specific food. This measurement takes into consideration how many grams of carbohydrate are in a specific food, and how much each gram raises blood sugar. For instance, 1 unit in glycemic load is equivalent to a 1 gram increase in blood glucose levels. Glycemic load is calculated based off a food’s glycemic index. More specifically, the glycemic load is the carbohydrate content of a specific food measured in grams (g), multiplied by the food’s GI, and divided by 100. It is worth noting that a food with a high glycemic index may not equate to a high glycemic load in our bodies.
Many weight loss diets take the glycemic index and glycemic load into consideration. However, the scientific evidence supporting such a dietary regimen is mixed. In general, it is better to consume all foods in moderation, and employ a healthy lifestyle. As always, before starting any diet, it is best to discuss your plans with your physician.